I can remember seeing images of SN1987A as it developed back in 1987. It was the explosion of a star, a supernova in the Large Magellanic Cloud. Over the decades that followed, it was closely monitored in particular the expanding debris cloud. Predictions suggested there may be a neutron star or even a black hole at the core but the resolution of the telescopes was insufficient to pick anything up. Now we have the James Webb Space Telescope and using its more powerful technology, signs of a neutron star have been detected.
Continue reading “Finally! Webb Finds a Neutron Star from Supernova 1987A”Could Forests Become Ultrahigh Energy Neutrino Detectors?
I really don’t know how to introduce this article. Neutrinos are elementary particles and are electrically neutral. They are produced by numerous cosmological events. Trees, well, we all know what they are and in a recent paper, scientists believed it may be possible to use entire forests as neutrino detectors! I was a bit sceptical when I read the paper but its an interesting concept and certainly trees have been used as broadband antennae so perhaps, well its a fascinating concept.
Continue reading “Could Forests Become Ultrahigh Energy Neutrino Detectors? “A New Observatory Will Spot Core-Collapse Supernovae Before They Explode
The thing about a supernova is that you never know when it might occur. Supernovae are triggered either by a collision with another star or when the interior of a massive star becomes depleted of nuclear fuel and begins a rapid collapse. Neither of these show any major optical changes before the explosion, so we are left to scan the sky in the hopes of catching one in its early stages. But that could soon change.
Continue reading “A New Observatory Will Spot Core-Collapse Supernovae Before They Explode”What are Leptons?
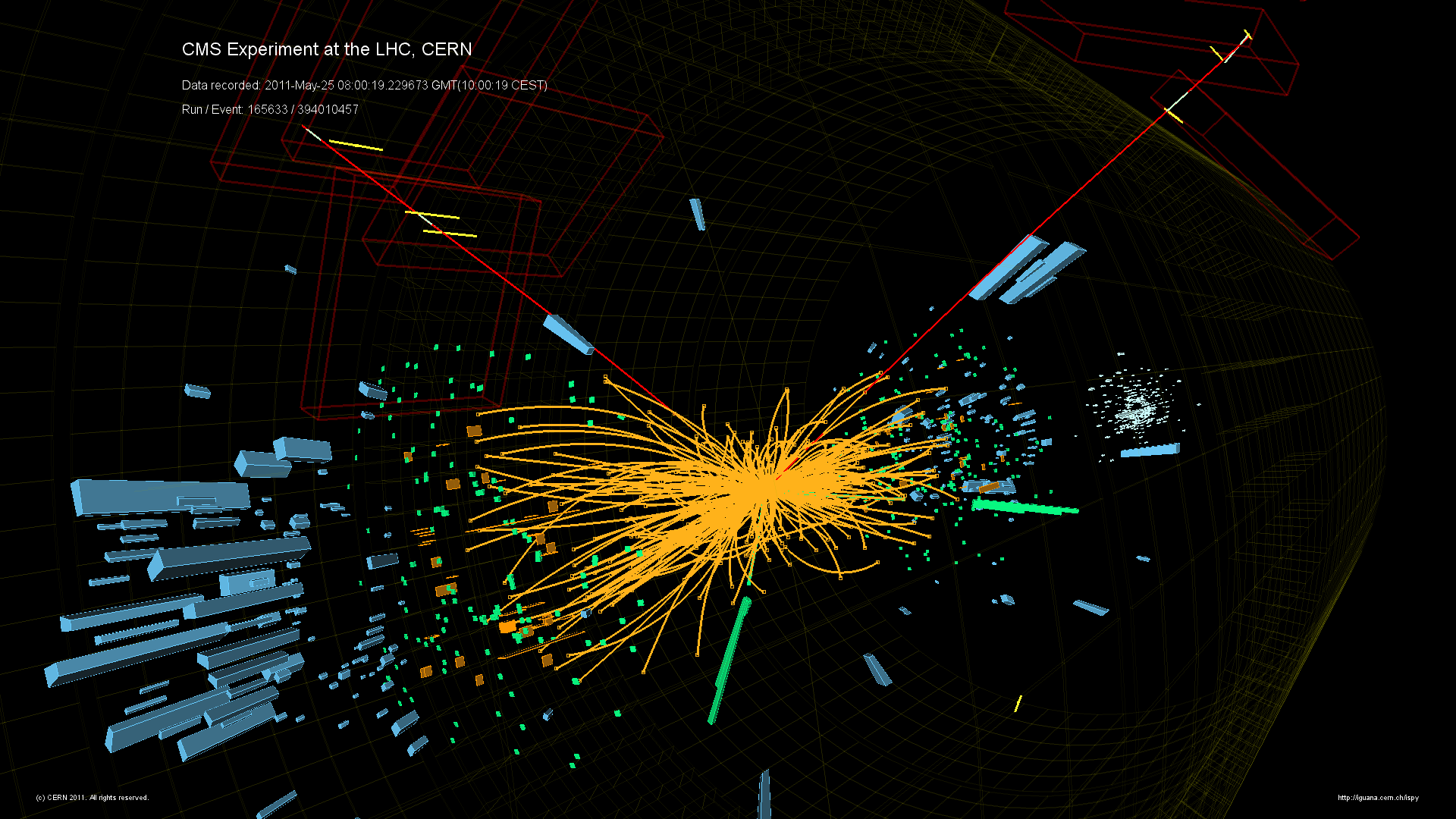
During the 19th and 20th centuries, physicists began to probe deep into the nature of matter and energy. In so doing, they quickly realized that the rules which govern them become increasingly blurry the deeper one goes. Whereas the predominant theory used to be that all matter was made up of indivisible atoms, scientists began to realize that atoms are themselves composed of even smaller particles.
From these investigations, the Standard Model of Particle Physics was born. According to this model, all matter in the Universe is composed of two kinds of particles: hadrons – from which Large Hadron Collider (LHC) gets its name – and leptons. Where hadrons are composed of other elementary particles (quarks, anti-quarks, etc), leptons are elementary particles that exist on their own.
Definition:
The word lepton comes from the Greek leptos, which means “small”, “fine”, or “thin”. The first recorded use of the word was by physicist Leon Rosenfeld in his book Nuclear Forces (1948). In the book, he attributed the use of the word to a suggestion made by Danish chemist and physicist Prof. Christian Moller.
Types of Leptons:
According to the Standard Model, there are six different types of leptons. These include the Electron, the Muon, and Tau particles, as well as their associated neutrinos (i.e. electron neutrino, muon neutrino, and tau neutrino). Leptons have negative charge and a distinct mass, whereas their neutrinos have a neutral charge.
Electrons are the lightest, with a mass of 0.000511 gigaelectronvolts (GeV), while Muons have a mass of 0.1066 Gev and Tau particles (the heaviest) have a mass of 1.777 Gev. The different varieties of the elementary particles are commonly called “flavors”. While each of the three lepton flavors are different and distinct (in terms of their interactions with other particles), they are not immutable.
A neutrino can change its flavor, a process which is known as “neutrino flavor oscillation”. This can take a number of forms, which include solar neutrino, atmospheric neutrino, nuclear reactor, or beam oscillations. In all observed cases, the oscillations were confirmed by what appeared to be a deficit in the number of neutrinos being created.
One observed cause has to do with “muon decay” (see below), a process where muons change their flavor to become electron neutrinos or tau neutrinos – depending on the circumstances. In addition, all three leptons and their neutrinos have an associated antiparticle (antilepton).
For each, the antileptons have an identical mass, but all of the other properties are reversed. These pairings consist of the electron/positron, muon/antimuon, tau/antitau, electron neutrino/electron antineutrino, muon neutrino/muan antinuetrino, and tau neutrino/tau antineutrino.
The present Standard Model assumes that there are no more than three types (aka. “generations”) of leptons with their associated neutrinos in existence. This accords with experimental evidence that attempts to model the process of nucleosynthesis after the Big Bang, where the existence of more than three leptons would have affected the abundance of helium in the early Universe.
Properties:
All leptons possess a negative charge. They also possess an intrinsic rotation in the form of their spin, which means that electrons with an electric charge – i.e. “charged leptons” – will generate magnetic fields. They are able to interact with other matter only though weak electromagnetic forces. Ultimately, their charge determines the strength of these interactions, as well as the strength of their electric field and how they react to external electrical or magnetic fields.
None are capable of interacting with matter via strong forces, however. In the Standard Model, each lepton starts out with no intrinsic mass. Charged leptons obtain an effective mass through interactions with the Higgs field, while neutrinos either remain massless or have only very small masses.
History of Study:
The first lepton to be identified was the electron, which was discovered by British physicist J.J. Thomson and his colleagues in 1897 using a series of cathode ray tube experiments. The next discoveries came during the 1930s, which would lead to the creation of a new classification for weakly-interacting particles that were similar to electrons.
The first discovery was made by Austrian-Swiss physicist Wolfgang Pauli in 1930, who proposed the existence of the electron neutrino in order to resolve the ways in which beta decay contradicted the Conservation of Energy law, and Newton’s Laws of Motion (specifically the Conservation of Momentum and Conservation of Angular Momentum).
The positron and muon were discovered by Carl D. Anders in 1932 and 1936, respectively. Due to the mass of the muon, it was initially mistook for a meson. But due to its behavior (which resembled that of an electron) and the fact that it did not undergo strong interaction, the muon was reclassified. Along with the electron and the electron neutrino, it became part of a new group of particles known as “leptons”.
In 1962, a team of American physicists – consisting of Leon M. Lederman, Melvin Schwartz, and Jack Steinberger – were able to detect of interactions by the muon neutrino, thus showing that more than one type of neutrino existed. At the same time, theoretical physicists postulated the existence of many other flavors of neutrinos, which would eventually be confirmed experimentally.
The tau particle followed in the 1970s, thanks to experiments conducted by Nobel-Prize winning physicist Martin Lewis Perl and his colleagues at the SLAC National Accelerator Laboratory. Evidence of its associated neutrino followed thanks to the study of tau decay, which showed missing energy and momentum analogous to the missing energy and momentum caused by the beta decay of electrons.
In 2000, the tau neutrino was directly observed thanks to the Direct Observation of the NU Tau (DONUT) experiment at Fermilab. This would be the last particle of the Standard Model to be observed until 2012, when CERN announced that it had detected a particle that was likely the long-sought-after Higgs Boson.
Today, there are some particle physicists who believe that there are leptons still waiting to be found. These “fourth generation” particles, if they are indeed real, would exist beyond the Standard Model of particle physics, and would likely interact with matter in even more exotic ways.
We have written many interesting articles about Leptons and subatomic particles here at Universe Today. Here’s What are Subatomic Particles?, What are Baryons?, First Collisions of the LHC, Two New Subatomic Particles Found, and Physicists Maybe, Just Maybe, Confirm the Possible Discovery of 5th Force of Nature.
For more information, SLAC’s Virtual Visitor Center has a good introduction to Leptons and be sure to check out the Particle Data Group (PDG) Review of Particle Physics.
Astronomy Cast also has episodes on the topic. Here’s Episode 106: The Search for the Theory of Everything, and Episode 393: The Standard Model – Leptons & Quarks.
Sources:
What Are The Parts Of An Atom?
Since the beginning of time, human beings have sought to understand what the universe and everything within it is made up of. And while ancient magi and philosophers conceived of a world composed of four or five elements – earth, air, water, fire (and metal, or consciousness) – by classical antiquity, philosophers began to theorize that all matter was actually made up of tiny, invisible, and indivisible atoms.
Since that time, scientists have engaged in a process of ongoing discovery with the atom, hoping to discover its true nature and makeup. By the 20th century, our understanding became refined to the point that we were able to construct an accurate model of it. And within the past decade, our understanding has advanced even further, to the point that we have come to confirm the existence of almost all of its theorized parts.
Shape-shifting neutrinos earn physicists the 2015 Nobel

What do Albert Einstein, Neils Bohr, Paul Dirac, and Marie Curie have in common? They each won the Nobel prize in physics. And today, Takaaki Kajita and Arthur McDonald have joined their ranks, thanks to a pioneering turn-of-the-century discovery: in defiance of long-held predictions, neutrinos shape-shift between multiple identities, and therefore must have mass.
The neutrino, a slight whiff of a particle that is cast off in certain types of radioactive decay, nuclear reactions, and high-energy cosmic events, could be called… shy. Electrically neutral but enormously abundant, half the time a neutrino could pass through a lightyear of lead without interacting with a single other particle. According to the Standard Model of particle physics, it has a whopping mass of zero.
As you can imagine, neutrinos are notoriously difficult to detect.
But in 1956, scientists did exactly that. And just a few years later, a trio of physicists determined that neutrinos came in not just one, not two, but three different types, or flavors: the electron neutrino, the muon neutrino, and the tau neutrino.
But there was a problem. Sure, scientists had figured out how to detect neutrinos—but they weren’t detecting enough of them. In fact, the number of electron neutrinos arriving on Earth due to nuclear reactions in the Sun’s core was only one-third to one-half the number their calculations had predicted. What, scientists wondered, was happening to the rest?
Kajita, working at the Super-Kamiokande detector in Japan in 1998, and McDonald, working at the Sudbury Neutrino Observatory in Canada in 1999, determined that the electron neutrinos were not disappearing at all; rather, these particles were changing identity, spontaneously oscillating between the three flavor-types as they traveled through space.
Moreover, the researchers proclaimed, in order for neutrinos to make such transformations, they must have mass.
This is due to some quantum funny business having to do with the oscillations themselves. Grossly simplified, a massless particle, which always travels at the speed of light, does not experience time—Einstein’s theory of special relativity says so. But change takes time. Any particle that oscillates between identities needs to experience time in order for its state to evolve from one flavor to the the next.
Kajita and McDonald’s work showed that neutrinos must have a mass, albeit a very small one. But neutrinos are abundant in the Universe, and even a small mass has a large effect on all sorts of cosmic phenomena, from solar nuclear physics, where neutrinos are produced en masse, to the large-scale evolution of the cosmos, where neutrinos are ubiquitous.
The neutrino, no longer massless, is now considered to play a much larger role in these processes than scientists had originally believed.
What is more, the very existence of a massive neutrino undermines the theoretical basis of the Standard Model. In fact, Kajita’s and McDonald’s discovery provided some of the first evidence that the Standard Model might not be as airtight as had been previously believed, nudging scientists ever more in the direction of so-called “new physics.”
This is not the first time physicists have been awarded a Nobel prize for research into the nature of neutrinos. In 1988, Leon Lederman, Melvin Schwartz, and Jack Steinberger were awarded the prize for their discovery that neutrinos come in three flavors; in 1995, Frederick Reines won a Nobel for his detection of the neutrino along with Clyde Cowan; and in 2002, a Nobel was awarded to Raymond David Jr., the oldest person ever to receive a the prize in physics, and Masatoshi Koshiba for their detection of cosmic neutrinos.
Kajita, of the University of Tokyo, and McDonald, of Queen’s University in Canada, were awarded the prestigious prize this morning at a news conference in Stockholm.
How are Energy and Matter the Same?
As Einstein showed us, light and matter and just aspects of the same thing. Matter is just frozen light. And light is matter on the move. How does one become the other?
Albert Einstein’s most famous equation says that energy and matter are two sides of the same coin.
But what does that really mean? And how are equations famous? I like to believe equations can be famous in the way a work of art, or a philosophy can be famous. People can have awareness of the thing, and yet never have interacted with it. They can understand that it is important, and yet not understand why it’s so significant. Which is a little too bad, as this is really a lovely mind bending idea.
The origin of E=mc2 lies in special relativity. Light has the same speed no matter what frame of reference you are in. No matter where you are, or how fast you’re going. If you were standing still at the side of the road, and observed a car traveling at ¾ light speed, you would see the light from their headlights traveling away from them at ¼ the speed of light.
But the driver of the car would still see that the light moving ahead of them at the speed of light. This is only possible if their time appears to slow down relative to you, and you and the people in the car can no longer agree on how long a second would take to pass.
So the light appears to be moving away from them more slowly, but as they experience things more slowly it all evens out. This also affects their apparent mass. If they step on the gas, they will speed up more slowly than you would expect. It’s as if the car has more mass than you expect. So relativity requires that the faster an object moves, the more mass it appears to have. This means that somehow part of the energy of the car’s motion appears to transform into mass. Hence the origin of Einstein’s equation. How does that happen? We don’t really know. We only know that it does.
The same effect occurs with quantum particles, and not just with light. A neutron, for example, can decay into a proton, electron and anti-neutrino. The mass of these three particles is less than the mass of a neutron, so they each get some energy as well. So energy and matter are really the same thing. Completely interchangeable. And finally, Although energy and mass are related through special relativity, mass and space are related through general relativity. You can define any mass by a distance known as its Schwarzschild radius, which is the radius of a black hole of that mass. So in a way, energy, matter, space and time are all aspects of the same thing.
What do you think? Like E=mc2, what’s the most famous idea you can think of in physics?
And if you like what you see, come check out our Patreon page and find out how you can get these videos early while helping us bring you more great content!
NOvA Experiment Nabs Its First Neutrinos
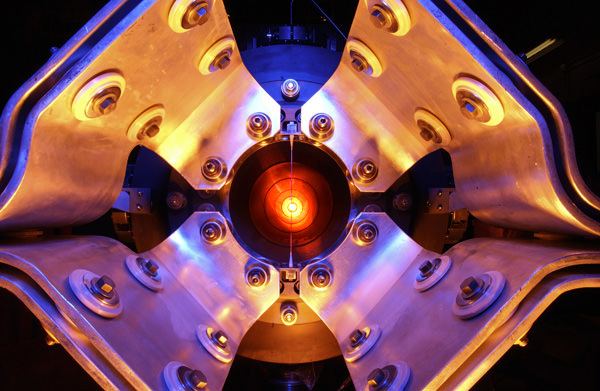
Neutrinos are some of the most abundant, curious, and elusive critters in particle physics. Incredibly lightweight — nigh massless, according to the Standard Model — as well as chargeless, they zip around the Universe at the speed of light and they don’t interact with any other particles. Some of them have been around since the Big Bang and, just as you’ve read this, trillions of them have passed through your body (and more are on the way.) But despite their ubiquitousness neutrinos are notoriously difficult to study precisely because they ignore pretty much everything made out of anything else. So it’s not surprising that weighing a neutrino isn’t as simple as politely asking one to step on a scale.
Thankfully particle physicists are a tenacious lot, including the ones at the U.S. Department of Energy’s Fermilab, and they aren’t giving up on their latest neutrino safari: the NuMI Off-Axis Electron Neutrino Appearance experiment, or NOvA. (Scientists represent neutrinos with the Greek letter nu, or v.) It’s a very small-game hunt to catch neutrinos on the fly, and it uses some very big equipment to do the job. And it’s already captured its first neutrinos — even before their setup is fully complete.
Created by smashing protons against graphite targets in Fermilab’s facility just outside Chicago, Illinois, resulting neutrinos are collected and shot out in a beam 500 miles northwest to the NOvA far detector in Ash River, Minnesota, located along the Canadian border. The very first beams were fired in Sept. 2013, while the Ash River facility was still under construction.
“That the first neutrinos have been detected even before the NOvA far detector installation is complete is a real tribute to everyone involved,” said University of Minnesota physicist Marvin Marshak, Ash River Laboratory director. “This early result suggests that the NOvA collaboration will make important contributions to our knowledge of these particles in the not so distant future.”
The beams from Fermilab are fired in two-second intervals, each sending billions of neutrinos directly toward the detectors. The near detector at Fermilab confirms the initial “flavor” of neutrinos in the beam, and the much larger far detector then determines if the neutrinos have changed during their three-millisecond underground interstate journey.
Again, because neutrinos don’t readily interact with ordinary particles, the beams can easily travel straight through the ground between the facilities — despite the curvature of the Earth. In fact the beam, which starts out 150 feet (45 meters) below ground near Chicago, eventually passes over 6 miles (10 km) deep during its trip.
According to a press release from Fermilab, neutrinos “come in three types, called flavors (electron, muon, or tau), and change between them as they travel. The two detectors of the NOvA experiment are placed so far apart to give the neutrinos the time to oscillate from one flavor to another while traveling at nearly the speed of light. Even though only a fraction of the experiment’s larger detector, called the far detector, is fully built, filled with scintillator and wired with electronics at this point, the experiment has already used it to record signals from its first neutrinos.”
The 50-foot (15 m) tall detector blocks are filled with a liquid scintillator that’s made of 95% mineral oil and 5% liquid hydrocarbon called pseudocumene, which is toxic but “imperative to the neutrino-detecting process.” The mixture magnifies any light that hits it, allowing the neutrino strikes to be more easily detected and measured. (Source)
“NOvA represents a new generation of neutrino experiments,” said Fermilab Director Nigel Lockyer. “We are proud to reach this important milestone on our way to learning more about these fundamental particles.”
After completion this summer NOvA’s near and far detectors will weigh 300 and 14,000 tons, respectively.
The goal of the NOvA experiment is to successfully capture and measure the masses of the different neutrino flavors and also determine if neutrinos are their own antiparticles (they could be the same, since they lack specific charge.) By comparing the oscillations (i.e., flavor changes) of muon neutrino beams vs. muon antineutrino beams fired from Fermilab, scientists hope to determine their mass hierarchy — and ultimately discover why the Universe currently contains much more matter than antimatter.
Read more: Neutrino Detection Could Help Paint an Entirely New Picture of the Universe
Once the experiment is fully operational scientists expect to catch a precious few neutrinos every day — about 5,000 total over the course of its six-year run. Until then, they at least now have their first few on the books.
“Seeing neutrinos in the first modules of the detector in Minnesota is a major milestone. Now we can start doing physics.”
– Rick Tesarek, Fermilab physicist
Learn more about the development and construction of the NoVA experiment below:
(Video credit: Fermilab)
Find out more about the NOvA research goals here.
Source: Fermilab press release
The NOvA collaboration is made up of 208 scientists from 38 institutions in the United States, Brazil, the Czech Republic, Greece, India, Russia and the United Kingdom. The experiment receives funding from the U.S. Department of Energy, the National Science Foundation and other funding agencies.
Polar Telescope Casts New Light On Dark Energy And Neutrino Mass
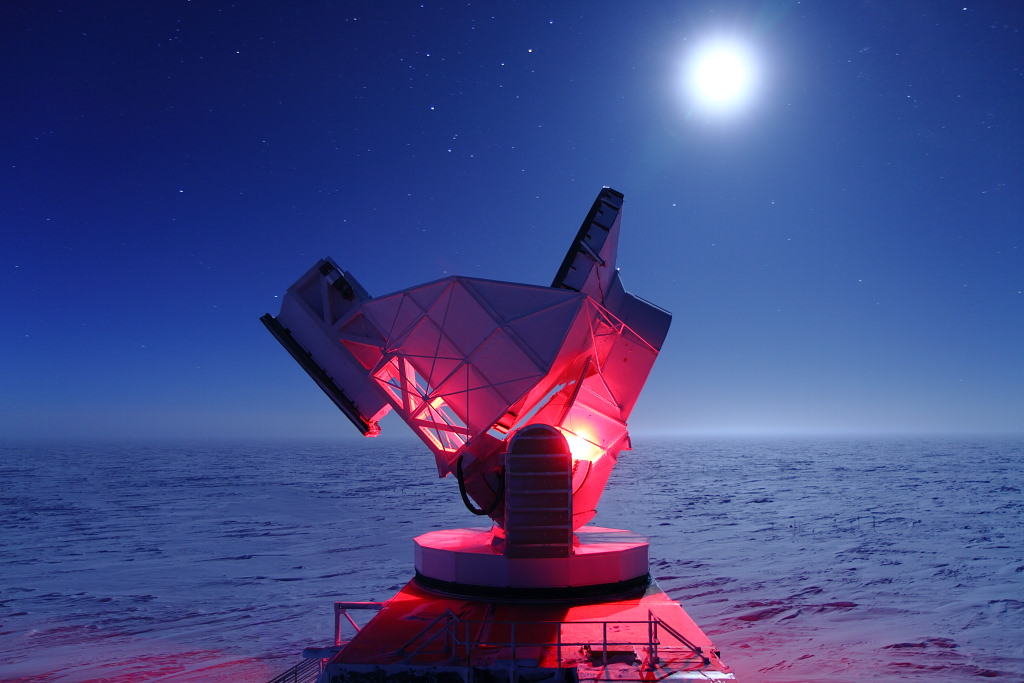
[/caption]
Located at the southermost point on Earth, the 280-ton, 10-meter-wide South Pole Telescope has helped astronomers unravel the nature of dark energy and zero in on the actual mass of neutrinos — elusive subatomic particles that pervade the Universe and, until very recently, were thought to be entirely without measureable mass.
The NSF-funded South Pole Telescope (SPT) is specifically designed to study the secrets of dark energy, the force that purportedly drives the incessant (and apparently still accelerating) expansion of the Universe. Its millimeter-wave observation abilities allow scientists to study the Cosmic Microwave Background (CMB) which pervades the night sky with the 14-billion-year-old echo of the Big Bang.
Overlaid upon the imprint of the CMB are the silhouettes of distant galaxy clusters — some of the most massive structures to form within the Universe. By locating these clusters and mapping their movements with the SPT, researchers can see how dark energy — and neutrinos — interact with them.
“Neutrinos are amongst the most abundant particles in the universe,” said Bradford Benson, an experimental cosmologist at the University of Chicago’s Kavli Institute for Cosmological Physics. “About one trillion neutrinos pass through us each second, though you would hardly notice them because they rarely interact with ‘normal’ matter.”
If neutrinos were particularly massive, they would have an effect on the large-scale galaxy clusters observed with the SPT. If they had no mass, there would be no effect.
The SPT collaboration team’s results, however, fall somewhere in between.
Even though only 100 of the 500 clusters identified so far have been surveyed, the team has been able to place a reasonably reliable preliminary upper limit on the mass of neutrinos — again, particles that had once been assumed to have no mass.
Previous tests have also assigned a lower limit to the mass of neutrinos, thus narrowing the anticipated mass of the subatomic particles to between 0.05 – 0.28 eV (electron volts). Once the SPT survey is completed, the team expects to have an even more confident result of the particles’ masses.
“With the full SPT data set we will be able to place extremely tight constraints on dark energy and possibly determine the mass of the neutrinos,” said Benson.
“We should be very close to the level of accuracy needed to detect the neutrino masses,” he noted later in an email to Universe Today.
Such precise measurements would not have been possible without the South Pole Telescope, which has the ability due to its unique location to observe a dark sky for very long periods of time. Antarctica also offers SPT a stable atmosphere, as well as very low levels of water vapor that might otherwise absorb faint millimeter-wavelength signals.
“The South Pole Telescope has proven to be a crown jewel of astrophysical research carried out by NSF in the Antarctic,” said Vladimir Papitashvili, Antarctic Astrophysics and Geospace Sciences program director at NSF’s Office of Polar Programs. “It has produced about two dozen peer-reviewed science publications since the telescope received its ‘first light’ on Feb. 17, 2007. SPT is a very focused, well-managed and amazing project.”
The team’s findings were presented by Bradford Benson at the American Physical Society meeting in Atlanta on April 1.
Underwater Neutrino Detector Will Be Second-Largest Structure Ever Built
[/caption]
The hunt for elusive neutrinos will soon get its largest and most powerful tool yet: the enormous KM3NeT telescope, currently under development by a consortium of 40 institutions from ten European countries. Once completed KM3NeT will be the second-largest structure ever made by humans, after the Great Wall of China, and taller than the Burj Khalifa in Dubai… but submerged beneath 3,200 feet of ocean!
KM3NeT – so named because it will encompass an area of several cubic kilometers – will be composed of lengths of cable holding optical modules on the ends of long arms. These modules will stare at the sea floor beneath the Mediterranean in an attempt to detect the impacts of neutrinos traveling down from deep space.
Successfully spotting neutrinos – subatomic particles that don’t interact with “normal” matter very much at all, nor have magnetic charges – will help researchers to determine which direction they originated from. That in turn will help them pinpoint distant sources of powerful radiation, like quasars and gamma-ray bursts. Only neutrinos could make it this far and this long after such events since they can pass basically unimpeded across vast cosmic distances.
“The only high energy particles that can come from very distant sources are neutrinos,” said Giorgio Riccobene, a physicist and staff researcher at the National Institute for Nuclear Physics. “So by looking at them, we can probe the far and violent universe.”
In effect, by looking down beneath the sea KM3NeT will allow scientists to peer outward into the Universe, deep into space as well as far back in time.
The optical modules dispersed along the KM3NeT array will be able to identify the light given off by muons when neutrinos pass into the sea floor. The entire structure would have thousands of the modules (which resemble large versions of the hovering training spheres used by Luke Skywalker in Star Wars.)
In addition to searching for neutrinos passing through Earth, KM3NeT will also look toward the galactic center and search for the presence of neutrinos there, which would help confirm the purported existence of dark matter.
Read more about the KM3NeT project here, and check out a detailed article on the telescope and neutrinos on Popsci.com.
Images property of KM3NeT Consortium