When astronomers first noted the detection of a Fast Radio Burst (FRB) in 2007 (aka. the Lorimer Burst), they were both astounded and intrigued. This high-energy burst of radio pulses, which lasted only a few milliseconds, appeared to be coming from outside of our galaxy. Since that time, astronomers have found evidence of many FRBs in previously-recorded data, and are still speculating as to what causes them.
Thanks to subsequent discoveries and research, astronomers now know that FRBs are far more common than previously thought. In fact, according to a new study by a team of researchers from the Harvard-Smithsonian Center for Astrophysics (CfA), FRBs may occur once every second within the observable Universe. If true, FRBs could be a powerful tool for researching the origins and evolution of the cosmos.
The study, titled “A Fast Radio Burst Occurs Every Second throughout the Observable Universe“, recently appeared in The Astrophysical Journal Letters. The study was led by Anastasia Fialkov, a postdoc researcher and Fellow at the CfA’s Institute for Theory and Computation (ITC). She was joined by Professor Abraham Loeb, the director of the ITC and the Frank B. Baird, Jr. Professor of Science at Harvard.
As noted, FRBs have remained something of a mystery since they were first discovered. Not only do their causes remain unknown, but much about their true nature is still not understood. As Dr. Fialkov told Universe Today via email:
“FRBs (or fast radio bursts) are astrophysical signals of an undetermined nature. The observed bursts are short (or millisecond duration), bright pulses in the radio part of the electromagnetic spectrum (at GHz frequencies). Only 24 bursts have been observed so far and we still do not know for sure which physical processes trigger them. The most plausible explanation is that they are launched by rotating magnetized neutron stars. However, this theory is to be confirmed.”
For the sake of their study, Fialkov and Loeb relied on observations made by multiple telescopes of the repeating fast radio burst known as FRB 121102. This FRB was first observed in 2012 by researchers using the Arecibo radio telescope in Puerto Rico, and has since been confirmed to be coming from a galaxy located 3 billion light years away in the direction of the Auriga constellation.
Since it was discovered, additional bursts have been detected coming from its location, making FRB 121102 the only known example of a repeating FRB. This repetitive nature has also allowed astronomers to conduct more detailed studies of it than any other FRB. As Prof. Loeb told Universe Today via email, these and other reasons made it an ideal target for their study:
“FRB 121102 is the only FRB for which a host galaxy and a distance were identified. It is also the only repeating FRB source from which we detected hundreds of FRBs by now. The radio spectrum of its FRBs is centered on a characteristic frequency and not covering a very broad band. This has important implications for the detectability of such FRBs, because in order to find them the radio observatory needs to be tuned to their frequency.”
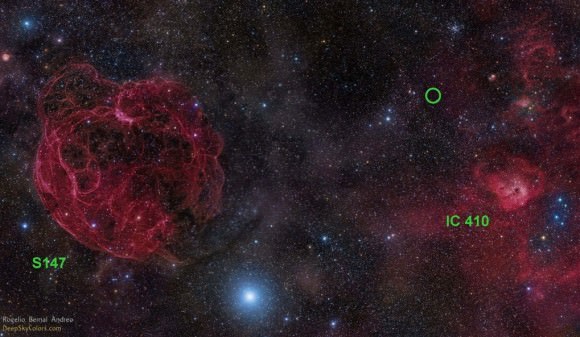
Based on what is known about FRB 121102, Fialkov and Loeb conducted a series of calculations that assumed that it’s behavior was representative of all FRBs. They then projected how many FRBs would exist across the entire sky and determined that within the observable Universe, a FRB would likely be taking place once every second. As Dr. Fialkov explained:
“Assuming that FRBs are produced by galaxies of a particular type (e.g., similar to FRB 121102) we can calculate how many FRBs have to be produced by each galaxy to explain the existing observations (i.e., 2000 per sky per day). With this number in mind we can infer the production rate for the entire population of galaxies. This calculation shows that an FRB occurs every second when accounting for all the faint events.”
While the exact nature and origins of FRBs are still unknown – suggestions include rotating neutron stars and even alien intelligence! – Fialkov and Loeb indicate that they could be used to study the structure and evolution of the Universe. If indeed they occur with such regular frequency throughout the cosmos, then more distant sources could act as probes which astronomers would then rely on to plumb the depths of space.
For instance, over vast cosmic distances, there is a significant amount of intervening material that makes it difficult for astronomers to study the Cosmic Microwave Background (CMB) – the leftover radiation from the Big Bang. Studies of this intervening material could lead to a new estimates of just how dense space is – i.e. how much of it is composed of ordinary matter, dark matter, and dark energy – and how rapidly it is expanding.

And as Prof. Loeb indicated, FRBs could also be used to explore enduring cosmlogical questions, like how the “Dark Age” of the Universe ended:
“FRBs can be used to measure the column of free electrons towards their source. This can be used to measure the density of ordinary matter between galaxies in the present-day universe. In addition, FRBs at early cosmic times can be used to find out when the ultraviolet light from the first stars broke up the primordial atoms of hydrogen left over from the Big Bang into their constituent electrons and protons.”
The “Dark Age”, which occurred between 380,000 and 150 million years after the Big Bang, was characterized by a “fog” of hydrogen atoms interacting with photons. As a result of this, the radiation of this period is undetectable by our current instruments. At present, scientists are still attempting to resolve how the Universe made the transition between these “Dark Ages” and subsequent epochs when the Universe was filled with light.
This period of “reionization”, which took place 150 million to 1 billion years after the Big Bang, was when the first stars and quasars formed. It is generally believed that UV light from the first stars in the Universe traveled outwards to ionize the hydrogen gas (thus clearing the fog). A recent study also suggested that black holes that existed in the early Universe created the necessary “winds” that allowed this ionizing radiation to escape.
To this end, FRBs could be used to probe into this early period of the Universe and determine what broke down this “fog” and allowed light to escape. Studying very distant FRBs could allow scientists to study where, when and how this process of “reionization” occurred. Looking ahead, Fialkov and Loeb explained how future radio telescopes will be able to discover many FRBs.
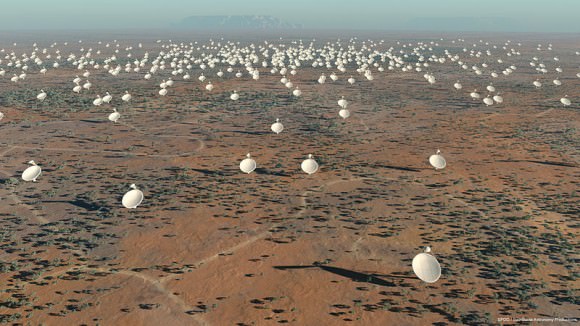
“Future radio observatories, like the Square Kilometer Array, will be sensitive enough to detect FRBs from the first generation of galaxies at the edge of the observable universe,” said Prof. Loeb. “Our work provides the first estimate of the number and properties of the first flashes of radio waves that lit up in the infant universe.”
And then there’s the Canadian Hydrogen Intensity Mapping Experiment (CHIME) at the at the Dominion Radio Astrophysical Observatory in British Columbia, which recently began operating. These and other instruments will serve as powerful tools for detecting FRBs, which in turn could be used to view previously unseen regions of time and space, and unlock some of the deepest cosmological mysteries.
“[W]e find that a next generation telescope (with a much better sensitivity than the existing ones) is expected to see many more FRBs than what is observed today,” said Dr. Fialkov. “This would allow to characterize the population of FRBs and identify their origin. Understanding the nature of FRBs will be a major breakthrough. Once the properties of these sources are known, FRBs can be used as cosmic beacons to explore the Universe. One application is to study the history of reionization (cosmic phase transition when the inter-galactic gas was ionized by stars).”
It is an inspired thought, using natural cosmic phenomena as research tools. In that respect, using FRBs to probe the most distant objects in space (and as far back in time as we can) is kind of like using quasars as navigational beacons. In the end, advancing our knowledge of the Universe allows us to explore more of it.
Further Reading: CfA, Astrophysical Journal Letters