Welcome back to our series on Exoplanet-Hunting methods! Today, we look at the curious and unique method known as Gravitational Microlensing.
The hunt for extra-solar planets sure has heated up in the past decade. Thanks to improvements made in technology and methodology, the number of exoplanets that have been observed (as of December 1st, 2017) has reached 3,710 planets in 2,780 star systems, with 621 system boasting multiple planets. Unfortunately, due to various limits astronomers are forced to contend with, the vast majority have been discovered using indirect methods.
One of the more commonly-used methods for indirectly detecting exoplanets is known as Gravitational Microlensing. Essentially, this method relies on the gravitational force of distant objects to bend and focus light coming from a star. As a planet passes in front of the star relative to the observer (i.e. makes a transit), the light dips measurably, which can then be used to determine the presence of a planet.
In this respect, Gravitational Microlensing is a scaled-down version of Gravitational Lensing, where an intervening object (like a galaxy cluster) is used to focus light coming from a galaxy or other object located beyond it. It also incorporates a key element of the highly-effective Transit Method, where stars are monitored for dips in brightness to indicate the presence of an exoplanet.
Description:
In accordance with Einstein’s Theory of General Relativity, gravity causes the fabric of spacetime to bend. This effect can cause light affected by an object’s gravity to become distorted or bent. It can also act as a lens, causing light to become more focused and making distant objects (like stars) appear brighter to an observer. This effect occurs only when the two stars are almost exactly aligned relative to the observer (i.e. one positioned in front of the other).
These “lensing events” are brief, but plentiful, as Earth and stars in our galaxy are always moving relative to each other. In the past decade, over one thousand such events have been observed, and typically lasted for a few days or weeks at a time. In fact, this effect was used by Sir Arthur Eddington in 1919 to provide the first empirical evidence for General Relativity.
This took place during the solar eclipse of May 29th, 1919, where Eddington and a scientific expedition traveled to the island of Principe off the coast of West Africa to take pictures of the stars that were now visible in the region around the Sun. The pictures confirmed Einstein’s prediction by showing how light from these stars was shifted slightly in response to the Sun’s gravitational field.
The technique was originally proposed by astronomers Shude Mao and Bohdan Paczynski in 1991 as a means of looking for binary companions to stars. Their proposal was refined by Andy Gould and Abraham Loeb in 1992 as a method of detecting exoplanets. This method is most effective when looking for planets towards the center of the galaxy, as the galactic bulge provides a large number of background stars.
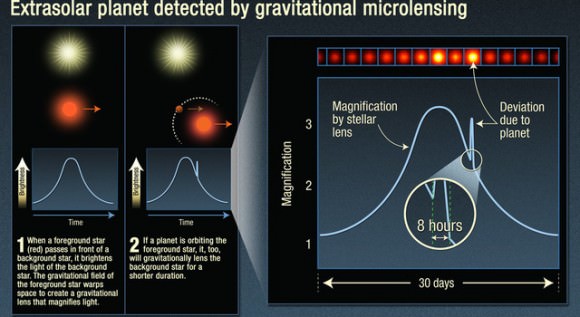
Advantages:
Microlensing is the only known method capable of discovering planets at truly great distances from the Earth and is capable of finding the smallest of exoplanets. Whereas the Radial Velocity Method is effective when looking for planets up to 100 light years from Earth and Transit Photometry can detect planets hundreds of light-years away, microlensing can find planets that are thousands of light-years away.
While most other methods have a detection bias towards smaller planets, the microlensing method is the most sensitive means of detecting planets that are around 1-10 astronomical units (AU) away from Sun-like stars. Microlensing is also the only proven means of detecting low-mass planets in wider orbits, where both the transit method and radial velocity are ineffective.
Taken together, these benefits make microlensing the most effective method for finding Earth-like planets around Sun-like stars. In addition, microlensing surveys can be effectively mounted using ground-based facilities. Like Transit Photometry, the Microlensing Method benefits from the fact that it can be used to survey tens of thousands of stars simultaneously.
Disadvantages:
Because microlensing events are unique and not subject to repeat, any planets detected using this method will not be observable again. In addition, those planets that are detected tend to be very far way, which makes follow-up investigations virtually impossible. Luckily, microlensing detections generally do not require follow-up surveys since they have a very high signal-to-noise ratio.
While confirmation is not necessary, some planetary microlensing events have been confirmed. The planetary signal for event OGLE-2005-BLG-169 was confirmed by HST and Keck observations (Bennett et al. 2015; Batista et al. 2015). In addition, microlensing surveys can only produce rough estimations of a planet’s distance, leaving significant margins for error.
Microlensing is also unable to yield accurate estimates of a planet’s orbital properties, since the only orbital characteristic that can be directly determined with this method is the planet’s current semi-major axis. As such, planet’s with an eccentric orbit will only be detectable for a tiny portion of its orbit (when it is far away from its star).
Finally, microlensing is dependent on rare and random events – the passage of one star precisely in front of another, as seen from Earth – which makes detections both rare and unpredictable.
Examples of Gravitational Microlensing Surveys:
Surveys that rely on the Microlensing Method include the Optical Gravitational Lensing Experiment (OGLE) at the University of Warsaw. Led by Andrzej Udalski, the director of the University’s Astronomical Observatory, this international project uses the 1.3 meter “Warsaw” telescope at Las Campanas, Chile, to search for microlensing events in a field of 100 stars around the galactic bulge.
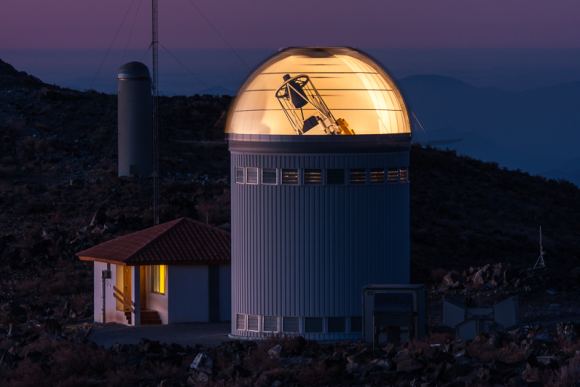
There is also the Microlensing Observations in Astrophysics (MOA) group, a collaborative effort between researchers in New Zealand and Japan. Led by Professor Yasushi Muraki of Nagoya University, this group uses the Microlensing Method to conduct surveys for dark matter, extra-solar planets, and stellar atmospheres from the southern hemisphere.
And then there’s the Probing Lensing Anomalies NETwork (PLANET), which consists of five 1-meter telescopes distributed around the southern hemisphere. In collaboration with RoboNet, this project is able to provide near-continuous observations for microlensing events caused by planets with masses as low as Earth’s.
The most sensitive survey to date is the Korean Microlensing Telescope Network (KMTNet), a project initiated by the Korea Astronomy and Space Science Institute (KASI) in 2009. KMTNet relies on the instruments at three southern observatories to provide 24-hour continuous monitoring of the Galactic bulge, searching for microlensing events that will point the way towards earth-mass planets orbiting with their stars habitable zones.
We have written many interesting articles on exoplanet detection here at Universe Today. Here is What are Extra Solar Planets?, What is the Transit Method?, What is the Radial Velocity Method?, What is Gravitational Lensing? and Kepler’s Universe: More Planets in our Galaxy than Stars
For more information, be sure to check out NASA’s page on Exoplanet Exploration, the Planetary Society’s page on Extrasolar Planets, and the NASA/Caltech Exoplanet Archive.
Astronomy Cast also has relevant episodes on the subject. Here’s Episode 208: The Spitzer Space Telescope, Episode 337: Photometry, Episode 364: The CoRoT Mission, and Episode 367: Spitzer Does Exoplanets.
Sources: